We are one step closer to achieve communication with those 37.2 trillion tiny components that make up our bodies. If you have ever wondered how the human body is capable of doing impressive amounts of chemical work without us even thinking about it, now you can understand it! Our bodies are efficient in converting energy, and communication among our cells is key to the understanding of all the basic processes that govern our life.
Cells often communicate via receptors made of sugars, that are exposed outside of their membranes. Such processes are often carried out by tiny sugar molecules that interact with those in their specific target. Recently, a team of researchers from The University of British Columbia published a synthetic method for modified sugars with incredible potential. In other words, it is now possible to obtain reliable materials to applications in cellular communication, metabolism and other biochemical processes.
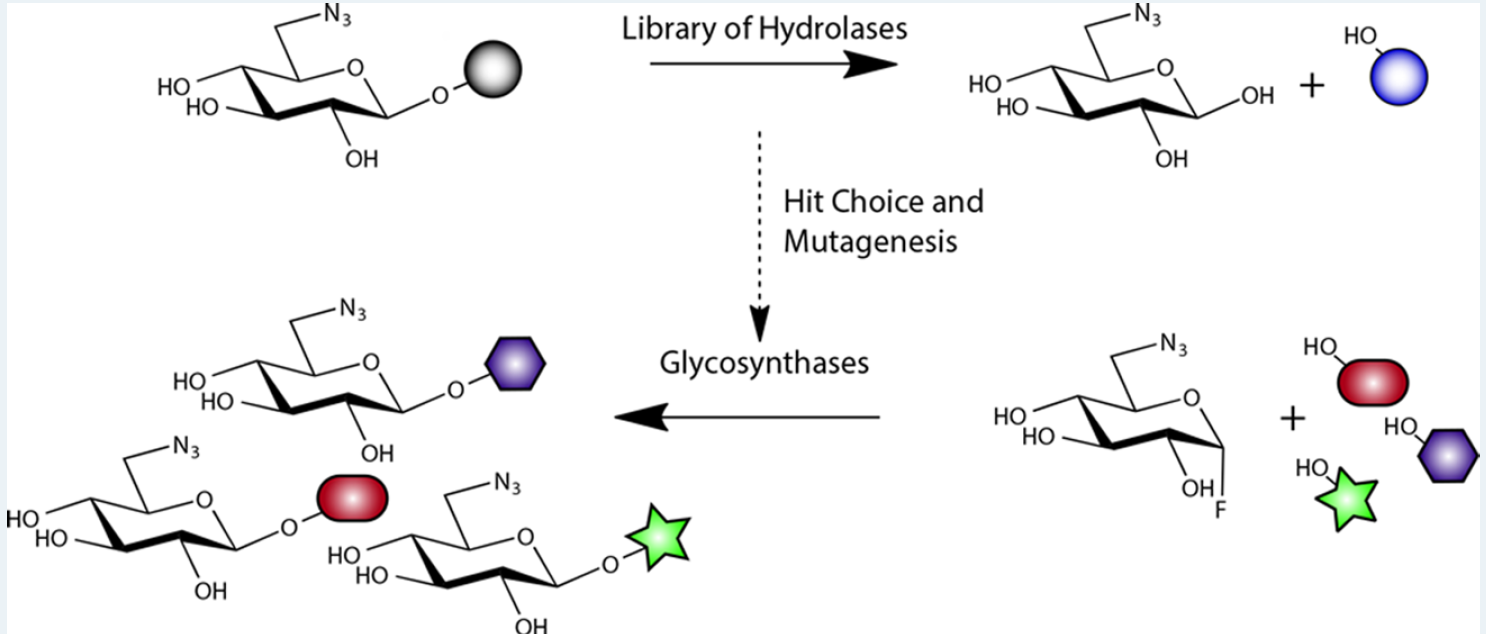
Figure 1: Structural representations of the transformation of sugars carried out by the researchers. Adapted from ACS Catalysis
This method was developed by using clones of genetically modified bacteria to express enzymes that are capable of modifying the sugar in accordance to the interest of the researcher. To achieve this, the scientists screened a library of 175 genes of the species E.coli that encoded variations of enzymes that can be used to catalyze selective chemical reactions in sugars for creating glycosidic bonds.
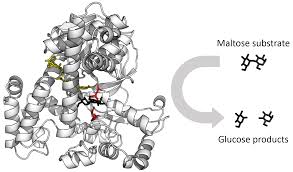
Computational representation of a hydrolase, an enzyme that breaks sugars. Adapted from Wikipedia
Enzymes are proteins that provide a path of a biochemical reaction to occur more efficiently. They are relatively easy to obtain and work with; however, they are specific to their target substrates which limit the extent in which their capabilities can be exploited. The scientists solved this issue by modifying the internal composition of the enzymes to improve the diversity of products in a process called selective mutagenesis. With the aid of this technique, the investigators obtained all variants that were tested in this experiment.
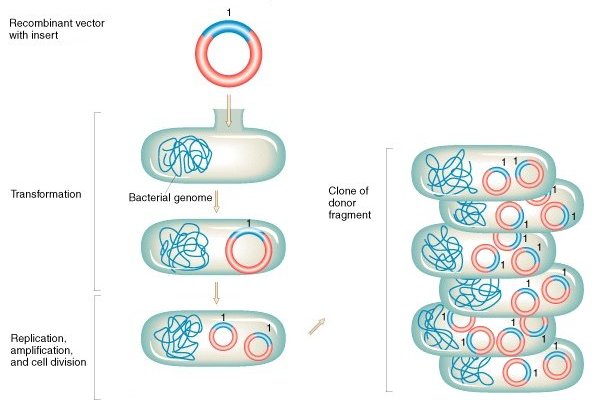
Schematic representation of bacterial transformation and cloning. Adapted from Griffiths et a (2000)
As the use of biotechnology increases, the understanding of our microscopic world becomes a major tool for scientific development. In this case, E.coli cells are essential since bacteria are inoculated with synthetic versions of genes that encode these enzymes and are then used as living machineries for protein production.
It is worth to mention that transformations of sugars were already reported. Nonetheless, previous methods rely on the use of expensive reagents as catalysts, which represent a major cost and are not widely accessible. This new approach opens a significant area in biochemical research. As technology improves to newer and more accessible methods, the diversification of these enzymes could develop new approaches for interaction with cell receptors that could enable us to understand what our cells have to say.
-Aron Engelhard
Journal Source:
Armstrong, Z.; Liu, F.; Chen, H.-M.; Hallam, S. J.; Withers, S. G. Systematic Screening of Synthetic Gene-Encoded Enzymes for Synthesis of Modified Glycosides. ACS Catalysis 2019, 9 (4), 3219–3227.